Scarcity Zero's technologies generate a lot of energy, but the driving mindset behind their deployment is their ability to cooperate by design. This concept – the idea of diverting the waste energy of one technology to help power the functions of another – is commonly referred to as “cogeneration,” or “Combined Heat and Power” (CHP). But it hasn't really been a central component of past power plant design, and has only recently started to gain prominence.[1] In general, our current power infrastructure just “there,” decentralized, ad-hoc systems that are each custom-designed and built to order. They rarely work together.[2] They barely talk to each other.[3] They are built with non-standardized components and powered by non-standardized fuels.[4] Just as importantly: the energy they generate is usually devoted only to powering electric generators, any excess is written off as “waste” instead of used for auxiliary or offsite functions.
This is a squandered opportunity on a monumental scale.
Most power plants today have an efficiency of around 33%.[5] This means 67% of their generated energy is discarded in the form of heat that either dissipates into the surrounding air or is absorbed into the ground. That’s more than twice the energy used to generate electricity in the first place. If we’re going to build an advanced and clean energy future, we must improve the efficiency and utility of our power infrastructure over the long-term.
The problem? Entropy and thermal loss are unavoidable byproducts of energy transfer – which means we’re probably never going to be able to build power plants that operate at superb levels of efficiency. But we can harness waste energy to power auxiliary functions at low additional cost. This is something Scarcity Zero seeks to employ at the design stage so that every aspect of power-generation is engineered to maximize cogeneration from the ground-up. And not just within a given facility, either, but also externally to other facilities that could be modularly integrated within an indefinitely scalable standard.
This writing commonly refers to these facilities as “CHP Plants” (Combined/Cogenerative Heat and Power), but their defining characteristic is simply a deployment of energy technologies designed to maximize efficiency and operational capability by leveraging waste energy for other useful applications.
While this design is significantly more aggressive than even our most ambitious approaches to energy efficiency, it is not inventive in and of itself, and has several proven demonstrations of its concepts within both power generation and manufacturing.[6] One notable example is the National Renewable Energy Laboratory’s REopt™ model (Renewable Energy Integration & Optimization), which is designed to identify renewable and/or energy efficiency opportunities within power infrastructure. One of their flagship projects in Arizona (home to the nation’s largest nuclear reactor)[7] involved the integration of light-water nuclear with renewables to efficiently desalinate seawater into hydrogen.[8]
Another example is China’s TSMR project in Gansu province that we reviewed in Chapter Four. Their cogenerative deployment leveraged up to 100 megawatts of clean energy to power resource-producing systems within fresh water, hydrogen fuel and chemical hydrocarbons.10 - [11]
Russia’s thorium project conducted by their School of Nuclear Science & Engineering of Tomsk Polytechnic University further leverages cogeneration to desalinate seawater into fresh water and hydrogen fuel.[13] Cogeneration has also been leveraged on varied scales with other energy technologies[14] and has been committed into official policies of the European Union[15] and the UK[16] that generally require its inclusion to participate in energy tax incentives.[17] The underlying benefit of cogeneration, therefore, is both proven and by itself isn’t especially novel as applies to energy generation.
But cogeneration today – even if applied to strong effect – still reflects the same foundational shortcomings of our current energy schema. Each effort looks for practical – yet piecemeal – improvements within unique, ad-hoc systems that are designed and implemented as such. Even if NREL’s REopt engineers retain the best tools and brightest minds in the world, everything they do for one application in one area will have to be completely re-done from scratch in another because the nature, capabilities, and limitations of the technology deployment hinge on energy systems that are essentially made to order.
By leveraging today’s manufacturing capabilities to mass-produce identical power-generating systems that are modular and standardized, Scarcity Zero seeks to promote an energy mindset that’s cogenerative-first. This means energy technologies are designed first – from blueprint to integration – to work cooperatively with others on a modular standard. That, then, makes the framework indefinitely scalable because identical power modules can be extended, integrated and/or swapped on-demand.
This helps change the fundamentals of the energy question from “how can we identify individual efficiency improvements within unique power-generating systems” to questions both more practical and expansive in vision.
For example, let’s say we were to ponder the following tasks:
- What would be required to build a power facility in mid-coastal California that generates 300 megawatts of electricity, desalinates 40 million gallons of seawater and produces two metric tons of hydrogen per day?
- With that known, how easily could we upgrade that facility’s desalination capacity to 60 million gallons, add on another ½ ton of hydrogen production and 100 megawatts more electricity?
- In case the upgrades in task #2 exceed an allocated budget, what deliverables would respectively meet 35% and 75% of this target?
- How much land would this require at what size of building envelope and what terrain limitations would apply?
- All aspects considered, what’s the overnight cost of these deployments with a high degree of certainty – plus or minus no more than 5% - all regulatory and permitting aspects considered?
In a world where energy systems are unique and made to order, the presence of these very questions sustains the business models of global consulting conglomerates. Their answers take months to derive and cost millions of dollars.
Yet in a world where capabilities of modular, mass-produced systems are known clearly, and their deployments both integrate and scale with others by design, answering these questions becomes far easier.
The unknowns are removed from the equation because each energy system is model-identical in similar application to a D-cell battery – and can couple, swap or decouple from others just like the very same. Everything from power requirements, performance specifications to product lifetime and physical footprint can be granulized in database tables that could be used to generate dynamic reports with the click of a mouse.
While the engineering complexities of each system of course remain, they’re also contained within the module. They do not permeate into the module’s capabilities or connection interfaces. Nor do they need to. Few of us know how to design or build a lightbulb, battery, USB hard drive or computer monitor, for instance.
We simply know how it’s supposed to work and how to replace it if it doesn’t.
And, if we want a second one, simply get a second one and plug it in. This is how the world of technology works in nearly every commercial sector. A rare exception is power-generation.
The time has come for that to change.
As applicable to Scarcity Zero, we’ll take a brief overview of how the concept of cogeneration would apply to CHP Plants.
Every technology within the framework deals with electricity, heat, and/or water. When these technologies are deployed in close proximity to one another, they can easily be tied together to harness waste energy.
The central technology behind CHP Plants are Liquid Fluoride Thorium Reactors (LFTRs). As far as power plants go, LFTRs get hot – quite hot – (900 °C / 1,600 °F).[18] As the reactor's heat exchangers are well-separated from anything radioactive and its electric generator is driven by an inert helium loop, their waste heat can be harnessed as central energy source for secondary functions.
The first function is seawater desalination.
Normally, a Multi-Stage Flash Distillation (MSFD) facility counterflows waste hot brine with cool seawater to preheat it for desalination, recycling energy in the process. But by directly integrating seawater intake with the heat exchangers of LFTRs beforehand, the seawater can be brought to a boil before it even reaches the facility. This increases efficiency in both systems and translates to cost savings. Further, it sterilizes any microbial life within the seawater – further preventing concerns with the aquatic transfer of invasive species.
The second function is hydrogen production, as there is already an ample supply of heat, electricity, freshwater and electrolyte (ocean salt) on site to extract hydrogen into fuel or base supplies for synthetic materials.
The third function is to use excess energy for atmospheric scrubbing of greenhouse gasses and air pollution, and as we’ll review later in Chapter Ten, waste processing via plasma gasification.
This becomes especially important in contexts of addressing climate change, because it is the most direct and effective tool we have available to physically remove greenhouse emissions already present in our atmosphere – and perhaps the most vital method available to reduce the circumstances fueling an inexorably warming planet.[19]
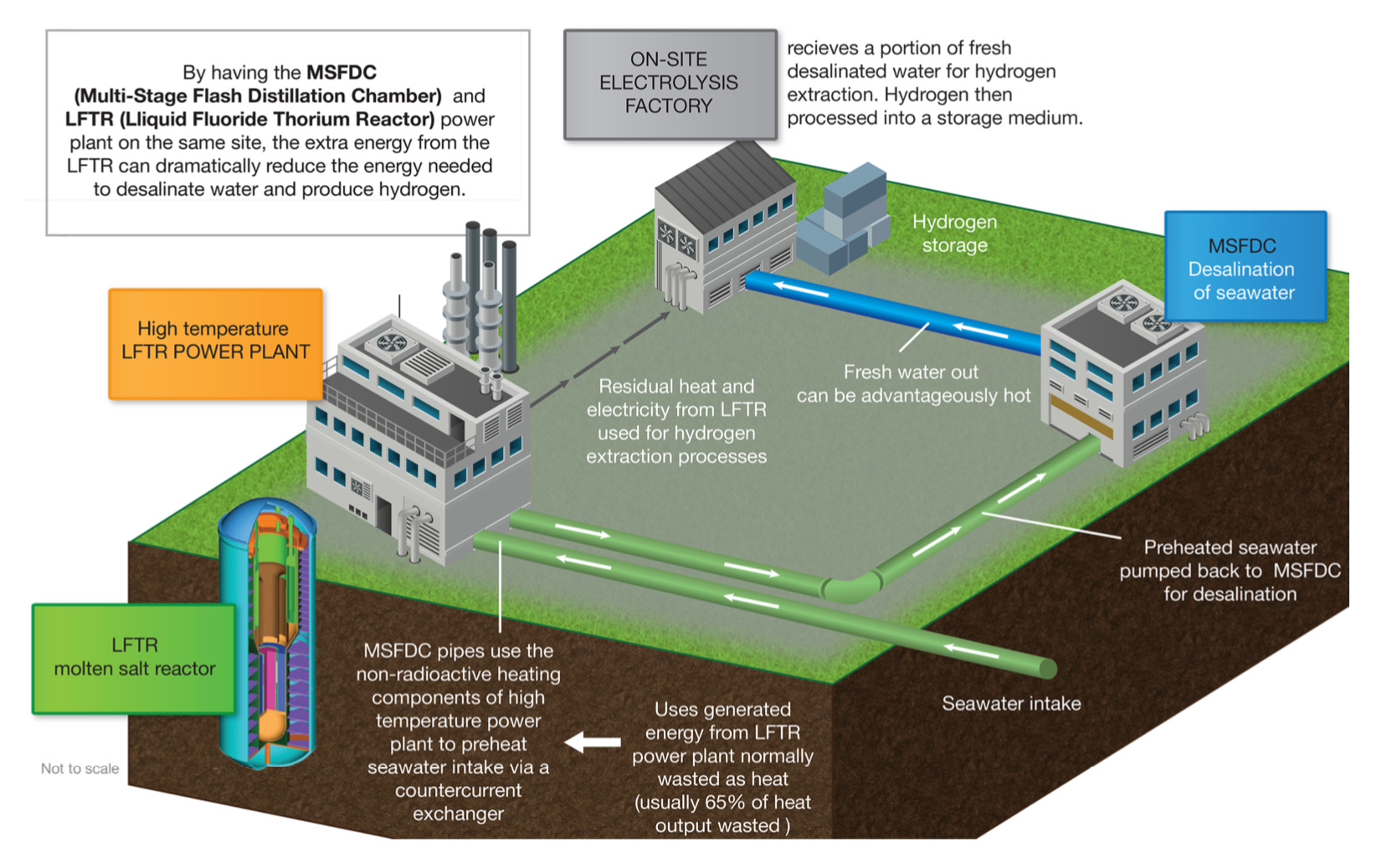
Atmospheric Scrubbing and Waste Processing
Climate change is a consequence of the concentration of greenhouse gases in our atmosphere. When the sun’s energy hits Earth, a portion of that energy is absorbed into the ground. Greenhouse gases, like carbon and methane, block more heat from radiating into space than nitrogen, which is the primary gas in earth’s atmosphere.[20] In function, this works much like a bedding blanket: the thicker the blanket, the more heat that blanket retains. The more heat it retains, the warmer you become – and this is happening on a planetary scale.[21] This problem adversely affects weather and long-term polar ice melt, but also exacerbates droughts, wildfires, smog and air pollution – saying nothing of mass human migration. Alongside resource scarcity, climate change ranks among the most serious problems of our time, with potentially catastrophic consequences for our civilization should it remain unaddressed.[22]
Worse, solutions to this problem that involve scaling back fossil fuels have proven politically precarious,[23] as the oil and gas industry is a major driver of the global economy and many entrenched power players owe their wealth to its lucrative returns. Even if this wasn’t the case, the greenhouse gas emissions from manufacturing, agriculture and global commerce would still persist even if our fuel supply chain was carbon neutral.[24]
Getting ahead of the impacts of climate change are laudable efforts. But they, in all truth, needed to begin decades ago when the first warnings were sounded (and unfortunately ignored).[25] Humanity has already passed an initial carbon tipping point of 400 parts-per-million[26] and global fossil fuel usage and consumption is accelerating[27] – as is our population – meaning that even though we recognize the problem of climate change, it’s getting continually worse even as we attempt to step up efforts to slow it down.
Switching to a clean, carbon-neutral energy schema like Scarcity Zero is an essential part of any strategy to avoid the calamitous results of climate change. But even if it was implemented as proposed it wouldn’t by itself clean the atmosphere of the greenhouse gasses already present. It can, however, power unique systems designed to accomplish this very task.
An atmospheric scrubber is a machine that strips greenhouse gases from the atmosphere, either by a chemical or mechanical method.[28] A primary example is Direct Air Capture, which blows air through towers containing a solution that reacts with certain compounds, removing them to form a substance that can be further processed into materials – including fuel.[29]
A Vancouver, Canada-based company named “Carbon Engineering” has patented several Direct Air Capture methods to isolate carbon from air through modular fan assemblies that work in unison with each other.[30] Their current pricing models assess a rate of $100 per-ton of CO2 captured under combined-cycle natural gas, which costs significantly more at present than Scarcity Zero’s target of 2 cents per kilowatt-hour.
As with other Direct Air Capture technologies, water and fuel are produced as deliverables alongside renewable electricity through cogenerative functions.
Another noteworthy component of this system is use of a chemical cycle that both uses non-toxic materials and is functionally closed-loop,[31] meaning that the chemicals (and thermal energy) used for Direct Air Capture is continually re-used and does not require frequent refueling over time. This makes the method both environmentally friendly and indefinitely scalable.
Several other ventures have come to market with similar technologies. Iceland’s Climeworks’ models, for example, are both modular and scalable with their largest units being capable of capturing nearly 5,000 kilograms of carbon per-day.[32] In addition to standard carbon capture, they also are able to condense captured carbon into usable fuels[33] or solid carbon for use in materials.[34]
Other companies, including Silicon Kingdom Holdings in the United Kingdom and Alabama-based Global Thermostat have obtained patents for similar models that perform similar functions in abstract.[35]
The costs of these systems stand to fall significantly over time through continued investment and research, and their operational costs stand to fall even further if implemented within a modular energy framework. And while cost reductions are realized maximally if integrated within CHP Plants, that shouldn’t overshadow other highly significant benefits that occur as well.
Because captured carbon is stored on-site, along with molecular hydrogen from electrolysis facilities, there is ample source material to make unique hydrocarbons with low-carbon emissions.[36] This means that not only are we able to produce hydrogen fuel through CHP Plants, we’re also able to further produce specialized hydrocarbons that have applications within chemical engineering, fuels for motor vehicles, heavy machinery, commercial aviation and aerospace.
Further, as any emissions from these applications (especially commercial aviation)[37] can be captured by these facilities and re-converted into usable fuel, we’re able to leverage the combined benefits of CHP Plants to not only help clean our atmosphere but present yet another avenue for sustainable fuel production.
Due to the modular nature of this approach, CHP Plants can be deployed both rapidly and at scale – also in a way that is location-agnostic. Building hundreds or thousands of them worldwide is limited only by vision and capital. Considering that their overnight cost is not prohibitively expensive[38] in the context of nationwide power[39] (or avoiding the consequences of resource scarcity and climate change), it’s an ideal approach that can cascade in effect to present immense social benefits practically anywhere on the planet.
In an ideal scenario, prefabricated facilities could be delivered turn-key, initiated in a matter of weeks for instant energy and resource production. With an effectively unlimited source of clean energy, problems of nearly any scale – even planetary – become solvable. And the more modular and adaptable these sources of energy become, the quicker they can arrive to deliver solutions that mitigate the impact of energy, climate or resource-driven social problems.
Symbiotic, cogenerative energy deployments are the next stage in our evolution of power generation not just because they solve several problems at once. They also address physical and economic challenges presented by our current ad-hoc approach to power generation:
- Constructing a single facility that can generate multiple types of energy and resources at one location is significantly less expensive than if these facilities were located far from each other.
- Consolidating multiple functions into a single facility avoids expenses of transmission and transportation, increasing overall efficiency.
- Symbiotic design helps establish ideal standards for implementation and operation. This reduces costs and helps encourage greater adoption of the Scarcity Zero framework.
- The fresh water produced from CHP Plants can come out hot – which will become important as we look at the concept of the National Aqueduct within the next few chapters.
What we can have with such symbiotic deployments of advanced energy technologies – what we can have today – is something that we have never before had in our history: the ability to synthetically, sustainably, and inexpensively produce as much electricity, water and fuel as we could ever need. All this while de-polluting our environment and combating climate change as a dedicated effort. And once we have these functions in hand, we can look beyond them, using any excess waste energy to produce resources to even greater extents.
While we began with electricity, water, and fuel out of necessity, we can extend the framework further in the fields of agriculture, chemical engineering, recycling processes and next-generation building materials. The next step towards that future comes from the National Aqueduct – a vital function of Scarcity Zero that ties each of its core technologies together into a nationwide network of energy and resource abundance.