The Scarcity Zero framework starts with renewable energy. Not just because it’s versatile and easily accessible, nor because it boasts a historically unprecedented capability to generate energy from a truly unlimited source. Rather, Scarcity Zero leverages renewables – specifically solar, wind and artificial hydroelectric – because of their ability to both integrate within large-scale infrastructure and power resource production as a municipal function.
This works across two areas of focus.
The first is the integration of solar and wind power directly within urban infrastructure to remove the obstacles and cost limitations they face today. This is a critically important distinction to our current approach towards solar and wind power, which only comprise 10% of energy generation nationwide.[1] While the usual suspects[2] in fossil energy lobbies certainly play a role in this,[3] the justified condemnation of their undue influence often glosses over the significant logistical and economic challenges to implementing renewables over a large scale. As we’ll see shortly, an integrational approach inherently avoids those challenges.
The second area of focus is The National Aqueduct, a vital function of Scarcity Zero intended to transport desalinated water anywhere in the country by piggybacking on the pre-cleared and largely publicly owned land of our interstate highway system and high-tension power line networks. Further, the National Aqueduct acts as both a power plant and a battery by deploying solar, wind, hydroelectric and thermoelectric functions as a single system. The National Aqueduct is detailed within Chapters Seven and Eight, but it’s useful to keep it in mind as it’s referenced several times before then.
As Scarcity Zero seeks, by design, to bypass the challenges inherent to renewable energy, we’ll take a minute to review these challenges and why they stand in the way of a clean energy future.
Location and transmission. Electricity, like sound, weakens over distance due to resistance in transmission mediums – in our case, power lines.[4] As a general rule, the farther electricity must travel, the harder it becomes to transmit. For example: there is enough open space in the American southwest for solar panels to power the entire planet.[5] Yet transmitting electricity from the southwest to locations thousands of miles away is both difficult and expensive. It’s possible to generate the energy, but we can’t efficiently get it from point A to point B once we start spanning large distances. Even if more efficient power lines emerged, they would still cost millions of dollars to build per-mile.[6] This means renewables are best deployed in locations close to where their generated energy is consumed.
Deployment expense and physical space. Solar and wind power require relatively large areas of land to be useful. Given that they are best deployed in locations close to energy consumption, this presents a secondary problem: land costs generally increase with population density. If land needs to be purchased for installation, the cost effectiveness of renewables proportionally reduces the closer they get to population centers.
While eco-conscious households and businesses can install renewables by choice, that option becomes harder for governments and power companies when they need to buy land at top dollar. So even as manufacturing costs of renewables continue to fall,[7] expenses of their start-to-finish implementation can remain quite high, both fiscally and politically.
Lack of standardization and prefabrication. As with many fledgling industries, our current approaches to solar and wind power remain unstandardized. Today, solar panels have extensive sizing options and are designed to be installed on varied locations: roofs, soil, rock, motorized platforms, etc. Wind turbines, likewise, come in all shapes and sizes for both residential and commercial applications. While flexibility in deployment is generally a good thing, there is not yet a modular and standardized method to implement renewables on a nationwide scale. This complicates the manufacturing of prefabricated, turnkey systems, increasing end unit cost and limiting overall usability.
Material throughput. Renewables require large volumes of materials to manufacture (10,000-17,000 metric tons per terawatt-hour),[8] materials that further need to be extracted, transported and processed in a carbon-emitting supply chain. This manufacturing process also carries its own drawbacks in terms of toxic waste.[9] Absent a massive carbon-neutral baseload power source such as thorium (outlined in Chapter Four), the large-scale manufacture and recycling of renewable energy and the costs therein would present tremendous carbon emissions and ecological toxicity – even if they themselves generate carbon-neutral energy.
These obstacles have slowed down the adoption of renewable power nationwide, and while advances in research and development have mitigated their impact to various degrees, they still remain substantial. Scarcity Zero seeks to solve this problem by targeting large-scale infrastructure for renewable deployment – especially public infrastructure.
Public infrastructure is the ideal location to install renewables, as it solves four of the five largest obstacles to their implementation: land, location, standardization and scale, with thorium solving the fifth (carbon-neutral manufacturing).
Here’s why:
No need to buy land. Municipalities can install solar and wind on publicly owned property by their own volition. They don’t need to submit bids or seek permission to buy expensive private land – cities can simply use city funds to deploy renewables on city-owned property to generate municipally offered electricity as they deem fit.
Close to population centers. In most cases, city-owned property tends to be close to cities. Public infrastructure, then, zeroes out the distance between generation and consumption. No expensive power lines need to be constructed, there is no need to transport electricity over long distances. And as advances in material science now allow us to build solar panels that are completely transparent (image to right), we don’t need to sacrifice aesthetics to take this approach.
Think of the streets in your city. The sides, windows and rooftops of every public or government building, every highway overpass and bridge, and every stadium built in part or full with public money; even privately-owned buildings. Imagine all of this infrastructure integrated with solar panels from the moment they were constructed – and all without spending so much as a nickel to buy extra land for their deployment. This image shows only one section of one area of one city.
Now imagine every city in America taking this approach. Applying this mindset across every municipality in the country gives us billions of square feet of surface space to deploy renewables that’s both close to consumption and doesn’t require us to buy expensive land.
Standardized manufacturing. Because renewables are so well-suited for large-scale infrastructure, this affords opportunities to build systems that can be mass-produced to a modular standard. Once you know exactly how something’s going to be implemented, it removes variables that add to unit cost and complexity – the end goal of any effective deployment strategy. That’s likely why several solar companies choose rooftops as locations for large-scale residential solar deployment: every home has a roof. The following images show Tesla corporation’s solar shingles, although several companies (RGS Energy, CertainTeed and SunTegra) have released similar products to market.[10]
As these surfaces both retain the aesthetics of traditional roofing tiles while boasting the functionality of solar panels, these companies identified a modular standard to deploy solar panels and manufactured their products to that standard. Yet they still require the personal investment of individual homeowners in order to adopt them on a large scale. By integrating renewables within municipal infrastructure, large-scale adoption can be accomplished with the stroke of a mayor’s pen. This incentivizes manufacturers to invest in renewable technologies that can be built to a standard for wide and consistent deployment nationwide.
Aside from the National Aqueduct, Scarcity Zero looks to three general types of large-scale infrastructure to deploy renewables. They include standing structures, road medians, and road canopies, which we’ll review in depth after taking a quick aside to make mention of some necessary assumptions.
A Quick Note:
The Next Giant Leap uses some terms surrounding energy that you might be a little unfamiliar with. We’ll take a minute to explain these terms (and their use in some basic arithmetic) more clearly to make it easier to grasp how Scarcity Zero works.
Energy vs. Power: Power quantifies the potential amount of energy that could be exerted in a given moment. Energy is the aggregate total of power exerted over time. Both power and energy are denoted in “watts,” with power denoted in-moment and energy over time. Watts is a metric unit of measure (1,000 watts = 1 kilowatt = 0.001 megawatt).
For example: if you turned on a 60-watt light bulb for ten seconds, that light bulb would have had 60 watts of power flowing through it. Yet it consumed 600 watt-seconds of energy (60 watts x 10 seconds). If it was on for an hour, it would have consumed 60 watt-hours (3,600 watt-seconds). If it was on for one year (8,760 hours) it would have consumed a total of 525,600 watt-hours of energy. That’s equivalent to 525.6 kilowatt-hours. At any given moment, though, it only had 60 watts of power flowing through.
Solar Power: In determining the effectiveness of solar power, we’re going to need to establish some baselines so we’re measuring consistently. Solar panels vary in size, sophistication and effectiveness, and there are several factors at play that determine both.
Peak sun hours is a unit of measure for solar panel generation capacity. It quantifies the aggregate total of solar energy that reaches a region in a day, denoted in hours of maximum solar intensity. Let’s say the sun shines for 13 hours a day over a region, depending on the season. That solar intensity may be equivalent to a certain number of “peak sun hours” of the sun at its maximum output (say at noon). Most regions of the country are exposed to an annual average of between 4.5-5.5 peak sun hours of solar energy per day. For our purposes here, we’ll split the middle and assume a total of five peak sun hours per day when doing our calculations for solar.
Solar panel output varies by sophistication, size, region and efficiency. So, we’ll have to establish some assumptions based on the myriad solar options available. The SunPower E20 solar panel is a solid quality solar panel available on the market today. With a nominal power output of 327 watts, it is 61.4 inches wide and 41.2 inches tall, coming to a surface area of about 17.5 square feet.[11] That translates to roughly 18.7 watts of power per square foot. Now let’s convert that to energy. If we circle back to the energy vs. power section on the prior page, 18.7 watts of power exerted over an hour is measured as 18.7 watt-hours. Assuming five peak sun hours in a day, that totals 93.5-watt hours generated per day. Extrapolated over a calendar year, that totals 34,127.5 watt-hours.
Denoted in kilowatt-hours (the standard most energy is measured in), that comes to 34.12 kilowatt-hours of energy generated per square foot, per year. To play it safe, however, we’ll cut that figure by 10%, and estimate that one square foot of solar panel surface can reliably generate 30 kilowatt-hours per year. As there are 27.88 million square feet in one square mile, we will conclude that one square mile of solar panels would reliably generate 836.5 million kilowatt-hours in a year.
Assumption Totals:
One square foot of solar generates: 30 kilowatt-hours per year.
One square mile of solar generates: 836.5 million kilowatt-hours per year.
According to the Energy Information Administration as of 2017, the average U.S. household consumes 10,400 kilowatt-hours per year.[12]
With these assumptions explained and these figures established, we’ll circle back to the revolutionary benefits of integrated renewables and how they can help build a clean energy future.
Standing Structures
Scarcity Zero’s first target for large-scale renewable deployment are standing structures with a primary emphasis on solar power. As we’ve invented solar panels that are both clear and visually unobtrusive, virtually any type of construction can be harnessed to generate clean energy from the sun.
This approach is ideal when it comes to location for solar deployment: standing structures are directly integrated within (and thus close to) population centers, and, as such, don’t require the purchase of additional land that might otherwise cost billions of dollars in aggregate. Further, in many cases, integrating solar can be accomplished as a seamless upgrade of the building’s existing features.
As a base concept, this idea isn’t new. Rooftops have been outfitted with solar panels since they were invented, and their use has increased as the costs of renewable energy have dropped over time. But recent advances in manufacturing and material science empower us to more deeply integrate solar into architectural designs. Solar glass now allows buildings to replace exterior windows with transparent solar panels. Solar-thermal HVAC uses excess solar energy to heat water and the building itself, generating electricity while at the same time slashing energy costs. Rooftop solar panels have never been cheaper, and for residential applications, solar shingles give homeowners the ability to use solar power with zero aesthetic sacrifice. Next-generation energy storage technologies tie all of the above together in a reliable, closed-loop system – an important feature, which we’ll review later in this writing.
When applied to existing buildings on the scale of skyscrapers or large office complexes, these advances can easily reduce energy use several times over – if not turn commercial buildings into mini power plants. To explain how, let’s circle back to windows. Consider the skyscraper to the left for a moment. Now imagine if every single one of those windows were replaced with solar glass – which again can be made aesthetically identical to normal glass. Just for one skyscraper, the energy potential would exceed hundreds of thousands of kilowatt-hours, even at moderate efficiencies – which would account for shadows or cloudy days as solar still works under such conditions. [13]
According to SolarWindow, a leading manufacturer of solar glass for commercial applications, a 50-story skyscraper in downtown Manhattan would generate 1.12 million kilowatt-hours annually if completely outfitted with solar windows (equivalent to the annual electricity usage of 108 single-family homes).[14] That number would rise to 1.38 million annually in Denver, and rise further to 1.57 million in Phoenix.[15] With present energy costs, the time it would take for each of those installations to pay for themselves is estimated to be roughly one year.[16] As solar glass can auto-tint based ambient brightness, the potential dividends for insulation and indoor climate management can be extended further.
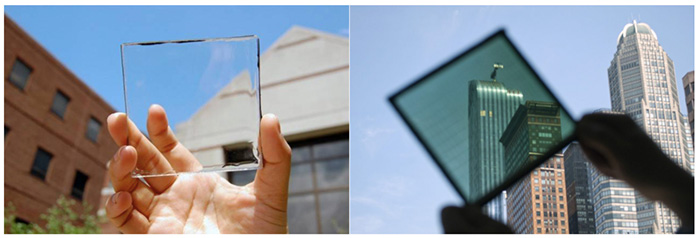
A large building such as the skyscraper above could likely generate more energy than it could reliably consume in a given day. What to do with this extra energy? It could be held locally in municipal storage (including the National Aqueudct), sold back to a local or municipal electric utility, or it could be diverted directly into its heating and cooling system.
The image to the right shows a basic diagram for a solar-thermal heating system. In concept, excess solar energy is used to heat water during the day, which is kept warm in an insulated storage tank. As water holds its temperature better than nearly any other substance, solar-heated water stored in insulated tanks dramatically reduces the need to use oil or natural gas for building heat. Notably, as solar panels are most efficient at colder temperatures,[17] this can save companies significant sums through reduced heating bills.
The benefits of upgrading existing buildings with solar windows and rooftop arrays of solar power will be limited primarily by the construction of the buildings themselves. To truly extend the capabilities of renewables on standing structures, integration could be considered by design at the architectural stage.
The following image shows the circular headquarters for PAF, a gambling management company owned and operated by the Finnish government. The building is wrapped completely in solar panels.
Another example is the Solar Valley Micro-E hotel in Northwest China, designed from the ground-up to maximize solar utility.
The Sanyo/Panasonic Solar Ark is a dual power plant and science museum dedicated to solar energy. Made from factory-rejected solar panels, it generates 500,000 kilowatt-hours of energy per year.[18]
These examples of solar buildings are prototypes, and, with the exception of the PAF headquarters in Finland, are initiatives undertaken by private companies. But these buildings act as solid proofs-of-concept, giving us ideas with which we can extend the integration of renewables within new structures.
Unlike passive solar design – an architectural trend from the 1970’s that attempted to design structures that would naturally take advantage of solar energy without the aid of mechanical equipment[19] – the sophistication of today’s technology and available commercial products in the renewable sector make “active solar designs” more straightforward. Technology can provide solar shingles, solar windows and solar walls at comparable or lower costs than fossilized energy sources.[20] Such technologies can further integrate through solar-enabled heating and air conditioning systems within a building. Managing a system could be accomplished through something as simple as a smartphone app.
Approaching architectural design with an eye to using active solar saves significant sums of energy and money. According to the U.S. Energy Information Administration, roughly 25% of a given commercial building’s total energy use was devoted to heating in 2012,[21] consuming the equivalent of some 556 billion kilowatt-hours of power in aggregate. At a rate of 10 cents per kilowatt-hour, that’s equivalent to $55.6 billion just to keep buildings heated – saying nothing of the electricity costs to keep the lights on and run equipment.
Every building that adopts an active solar system avoids unnecessary energy expenses and further presents less demand on the local electrical grid. Each building further helps create a cascading effect of increasing the amount of energy generated while decreasing total energy consumption.
This cascading effect becomes important when other infrastructure is selected for potential solar energy deployment. Take bridges for example.
The Sunshine Skyway Bridge in Tampa, for example, is 4.14 miles long (roughly 21,900 feet).[22] If we were to assume an average of only 40’ of deployment surface on each side of the 430-foot tall structure, the Skyway bridge would feature 1.75 million square feet of surface area that could be mounted with clear, aesthetically unobtrusive solar panels. When we shortly consider the implications of solar road canopies, the 94’ wide driving surface would add another 2.05 million square feet of surface area to a total of 3.81 million.
As one square foot of solar panels can reliably generate 30 kilowatt-hours annually in areas with solid sun exposure, the Skyway bridge could passively generate 114 million kilowatt-hours of electricity per year without having to buy a single piece of land – enough to power nearly 11,000 homes.
Another promising example of usable infrastructure is the parking lot. Our nation is littered with parking lots that are flat and open:
As they’re dark in color, they absorb lots of heat, and anyone who drives in summer knows full well that they turn cars to ovens – presenting serious safety risks to children or pets left inside. This makes parking lots uniquely well suited for solar panels, a concept that’s been increasingly adopted as solar panel prices have dropped.
Solar parking lots solve several problems at once. They generate electricity that can be used to power the building that owns the lot; charge the electric cars of customers or employees; or sell energy back to a local or municipal electric grid. Solar canopies further provide shade that keeps cars cool. Across an urban landscape, solar parking lots could generate a massive amount of electricity that could further increase our total generating capacity (and thus supply) while reducing demand from external energy sources.
Assuming a parking lot of 150,000 square feet (roughly equivalent to a local Lowe’s),[23] sticking to the figure of 30 kilowatt-hours of electricity generated per square foot, per year, that parking lot would generate roughly 4.5 million kilowatt-hours annually. If for sake of argument, the local Target, Home Depot, Walmart, Costco and Sam’s all had the same footprint, the combined total would be 27 million kilowatt-hours annually generated. That’s enough to power more than 2,500 homes. It’s plain, then, to see how these numbers can add up quickly over large areas of urban sprawl. This becomes even more true once road medians and solar road canopies enter the equation.
Road Medians
As one of the greatest publics works projects of the 20th century, highways present uniquely attractive locations to install renewable energy. They’re completely cleared of obstructions, and as they’re generally flat and straight, they get extensive sun and wind exposure. They’re often close to cities, almost always municipally owned, and easily accessible.
While roads themselves can also be extended to integrate solar power via overhead canopies, highways often feature medians – unpaved barriers between lanes of traffic (especially on city outskirts) – which are perfect locations to deploy renewable energy. Like the highway itself, medians are usually municipally owned as well. Yet they serve no functional purpose apart from separating directional traffic. Additionally, they require constant maintenance (mowing, landscaping, etc.), which comes with significant additional costs. So, why not repurpose them for solar panels and wind turbines, solving two problems at once? Japan, South Korea, China and The Netherlands have done exactly that:
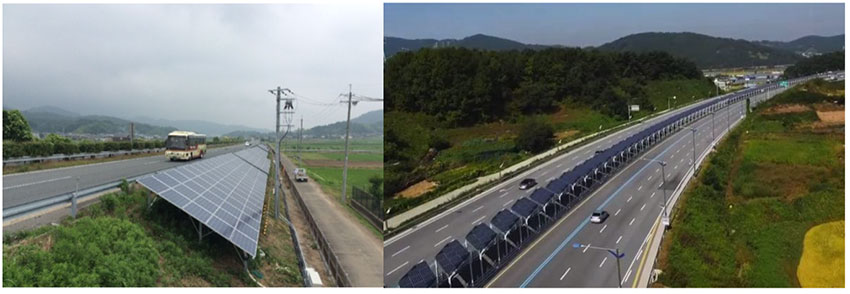
In the case of South Korea, their solar road median also doubles as a covered bike path for non-motorized commuters (image above).
This approach provides several benefits. First, sun exposure is completely maximized. Unless the sky itself is overcast, sunlight will reach these panels from the moment it rises until the moment it sets – and solar still works even when it’s cloudy.[24] Since highways span thousands of miles in aggregate, the surface area presented by employing medians is tremendous – surface area that’s within the ideal distance for maximum effectiveness of renewables. This approach is also easy. Highway medians are common and simple to access, yet serve little utility in their current form. Repurposing them as solar farms creates massive utility by using publicly-owned land – land that would otherwise cost billions at fair market value – to generate energy.
Further, there’s no reason why wind turbines couldn’t be installed above solar panels at regular intervals. They cast minimal shadow, present no risk to motorists, and can complement electricity generation twenty-four hours a day. The ideal height, size and shape of wind turbines (either horizontal or vertical axis, as shown by the images below) is debatable, but their utility is clear. And, when installed within medians, they also come with zero overhead land cost.
Installing renewables in highway medians could be a simple extension of municipal highway management. All a work crew would need to do to install, repair or replace a section of these panels or turbines is simply drive up to them and perform the required work – just as they would with any road maintenance today. Power lines could be run in modular conduit channels under solar panels and connect to municipal electric grids. The scale of implementation could be increased piecemeal, as funding allowed, to be extended indefinitely so long as there is available median surface.
Just as importantly, as this energy is generated by the municipality, it can be sold by the municipality – costing significantly less than if sold by a for-profit utility company. This further provides an extra revenue stream which cities can use to extend their service offerings or fund other initiatives outside of standard municipal budgets.
These side benefits, however, aren’t the central focus. A one mile-stretch of solar arrays (at ten feet wide) would comprise 52,800 square feet of surface, which at 30 kilowatt-hours generated per square foot annually would arrive at a combined total of 1.58 million kilowatt-hours – enough to power 150 homes. Any energy generated by wind turbines would be supplementary. There are approximately 4 million miles of public roads in the United States.[25] Yet while not all road surfaces boat medians (or proximity to power consuming locations), it does present attractive opportunities to install overhead solar canopies to generate energy – especially within cities.
Solar Road Canopies
At concept, a solar road canopy is simply a rectangular canopy over a road surface that’s built on pylons. As roads are generally flat, straight and cleared at the sides from overhead obstructions, they’re another easy way to deploy millions of solar panels. The four million miles of American road surfaces – some 8.3 million lane-miles[26] – are among the most comprehensive in the world; they connect every city in the nation together and cover thousands of square miles – some 18,860 if we were to assume each lane of road had the national average width of 12 feet.[27]
Extrapolated into square feet, that’s a cool 525.89 billion. At 30 kilowatt-hours per square foot, per year, even one-tenth of that area would output 1.58 trillion kilowatt-hours – roughly half our national energy consumption. Further, roads also remain municipally owned, which like highway medians and public infrastructure allows for large-scale deployment while avoiding the need to purchase expensive land. Considering the map below, how many other public locations do we have available – at such size or interconnectivity – to deploy a nationwide network of anything to a single standard? Roads are the zenith for integrating renewables within large-scale infrastructure.
However, power is not the only benefit presented by solar road canopies. Heat management is another major aspect – especially within cities. As summer months continually increase in temperature through the encroaching impact of climate change,[28] cities are finding it ever-more challenging to deal with conditions hot enough to melt asphalt.[29] Placing solar canopies over roads would perform the same effect as placing solar canopies over parking lots – providing shade, keeping the surface below cool and reflecting heat upward instead of having it absorbed into the ground.
Another important aspect is the potential for stormwater management. Water management on roads is a major consideration of both safety[30] and environmental impact.[31] By integrating gutter systems into the solar canopies, such canopy arrays can divert water into nearby water management systems instead of letting it fall on road surfaces. In the context of drier climates with limited rainfall, this can present a highly attractive way to replenish municipal water supplies – especially if solar canopies are deployed over a larger scale. In the context of the National Aqueduct, this presents an especially effective method to deliver auxiliary water as well as store solar energy generated during the day – helping keep roads drier and safer during storms.
While solar road canopies would generate immense energy over distance, further benefits could include integration with road technology aids. Heating elements to keep panels clear of snow, municipal WiFi networks, wireless charging for electric vehicles, directional GPS guidance and auxiliary wireless guidance for autonomous vehicles are all examples in this respect.
As all canopies would require in abstract are metal pylons, a cross-lattice to install panels, wiring and plumbing, the overall deployment expenses and logistical challenges thereof are significantly limited – all the more since they would be installed on municipal property and thus spared of land costs.
What About Storage?
Applying solar and wind power to standing structures, highway medians, and road canopies integrates renewable energy into municipal infrastructure, creating a self-reinforcing system that’s easy to scale and maintain over time. The next question is storage: how can we keep the energy generated by renewables for later use? Solar doesn’t work at night and works less efficiently during overcast days. Wind power only works when the ambient environment is windy. Renewables, therefore, will only be as effective as their ability to pair with storage mediums. This has proven significantly challenging – and expensive – for large-scale renewable deployment in the past, and has presented risks to energy availability at times of unexpected demand.[32]
The National Aqueduct (which we’ll go over in Chapter Seven) is the primary method Scarcity Zero employs to solve this problem within the framework. But there are several other promising energy storage mediums that can work on a smaller scale when needed – especially in remote locations.
One option comes from Tesla. Their flagship battery products: the Tesla Powerwall (home use) and Powerpack (commercial applications), provides piecemeal solar storage solutions at relatively modest costs.
On a commercial scale, Powerpack units can work together, in parallel, to maximize energy storage capacity. As each unit has a storage capacity of up to 200 kilowatt-hours, an array of hundreds could easily store the energy needed for most municipal applications.
Liquid-metal batteries, such as the ones sold by Ambri corporation, provide another option for mass power storage. Unlike lithium-ion batteries used by Tesla and most commercial electronics, liquid-metal batteries use a combination of magnesium and antimony suspended within a liquid salt electrolyte to store electricity.[33] These materials are highly abundant and accessible, and modular battery designs allow for straightforward manufacturing. As their battery arrays are intended to be installed within standard-sized shipping containers, they enable flexible deployment strategies to provide instant-on capability to any electric grid in the world.
Scientists in Beijing recently discovered how to make batteries from potassium-ion,[34] which provides ample supplies from seawater.[35] These batteries are especially promising because they do not require the often environmentally destructive practices of lithium and cobalt mining, yet also boast attractive features within energy density and charging capacity. The current prototypes retain 90% of their energy storage capacity after 10,000 charging cycles, which shows significant potential for a technology in its early infancy.[36]
A fourth viable option is heat. As opposed to storing electricity directly, thermal energy storage systems capture heat that can be converted into electricity via thermoelectric features. Some of the most prominent options on the market today are molten salt batteries that store heat energy in a salt which doubles as an electrolyte, efficiently enabling high-energy output.[37] Solar-thermal troughs and hydro-solar thermal arrays use solar energy to generate excess heat for future conversion into electricity[38] – by itself a core function of the National Aqueduct.
These advances in commercial battery technology are effective options designed for use at a municipal scale. Implemented as such, they can help sustain critical city-wide systems or provide backup functionality for municipal power grids in the event of blackouts. As their scale increases over time, they can provide expanded storage functionality for renewable energy until the point where the municipality becomes self-sustaining. This becomes all the more possible once cities are connected to the National Aqueduct.
It’s important to reemphasize that these technologies and the commercial products they reflect are still in their infancy, and haven’t yet scratched the surface of their future potential. As with the first car, aircraft or smartphone, new technologies advance over time through increased economies of scale, future investments in research and development, manufacturing efficiency, and consumer feedback. Their cost and performance today are not what it could be tomorrow, next year, or in the next decade.
But even in their infancy, these renewable energy sources, when integrated into large-scale infrastructure and combined with next-generation commercial energy storage, complete a circle. Deploying renewables in cities transforms their capabilities by maximizing their scale while minimizing costs. Once complete, this circle sets the stage for an evolution in city design that dramatically expands the potential of the contemporary and future metropolis. Cities, now net energy consumers, can one day become net energy producers with increased potential to improve quality of life for their inhabitants. This change is the key to the cities of tomorrow, built by our hand today.