Of the resources facing impending scarcity, water and fuel are especially important as they respectively make life possible and power vital aspects of our advanced economy. They are also critical to growing, processing and delivering food. Consequently, these resources are the most likely to drag us into conflict when they run low – which is unsurprisingly why we've been fighting over them throughout much of history. Scarcity Zero seeks to solve this problem by generating enough energy to synthesize these resources, further enabling us to produce synthetic building materials on an effectively unlimited scale.
With renewables and thorium in place, the next focus is seawater.
It’s important to note in this context that humanity isn’t facing a water crisis in abstract; we’re facing, specifically, a freshwater crisis. 71% of the planet’s surface is covered by water, yet less than 2% of that water is fresh – and 80% of that freshwater is locked in polar ice.[1] For consumption purposes, that last 20% of freshwater - 0.4% of all water on our planet – is the only percentage that has historically mattered. Thanks to modern seawater desalination technologies, that is no longer true today.
The desalination of seawater is a well-proven concept.[2] The same is true of extracting hydrogen fuel from water via electrolysis, as running an electric current through water chemically separates it into hydrogen and oxygen.[3] Yet both processes require lots of energy, which has traditionally made each of them expensive. The energy generated by LFTRs and municipally integrated renewables removes energy cost as a major factor, allowing us to extract fresh water and hydrogen fuel from seawater on a massive scale.
This begins with a system known as a Multi-Stage Flash Distillation Chamber (MSFD),[4] as seen in this diagram:
An MSFD facility features a series of interconnected chambers (referred to as “stages”) set at varied temperatures and pressures relating to the boiling point of water. Seawater is pumped in through one end and heated to reach a certain temperature. Once at the right temperature, it’s then pumped into subsequent stages, each of which has a different temperature and pressure. This process forces seawater to instantly flash-turn to steam, which is then collected via a condenser and turned into liquid fresh water.
From there, the remaining hot brine is pumped back into the system to counterflow with the influx of cold seawater, helping to heat new seawater and recycle a majority of heat energy in the process.[6] What waste remains is essentially very salty water, which can be evaporated to leave only salt.
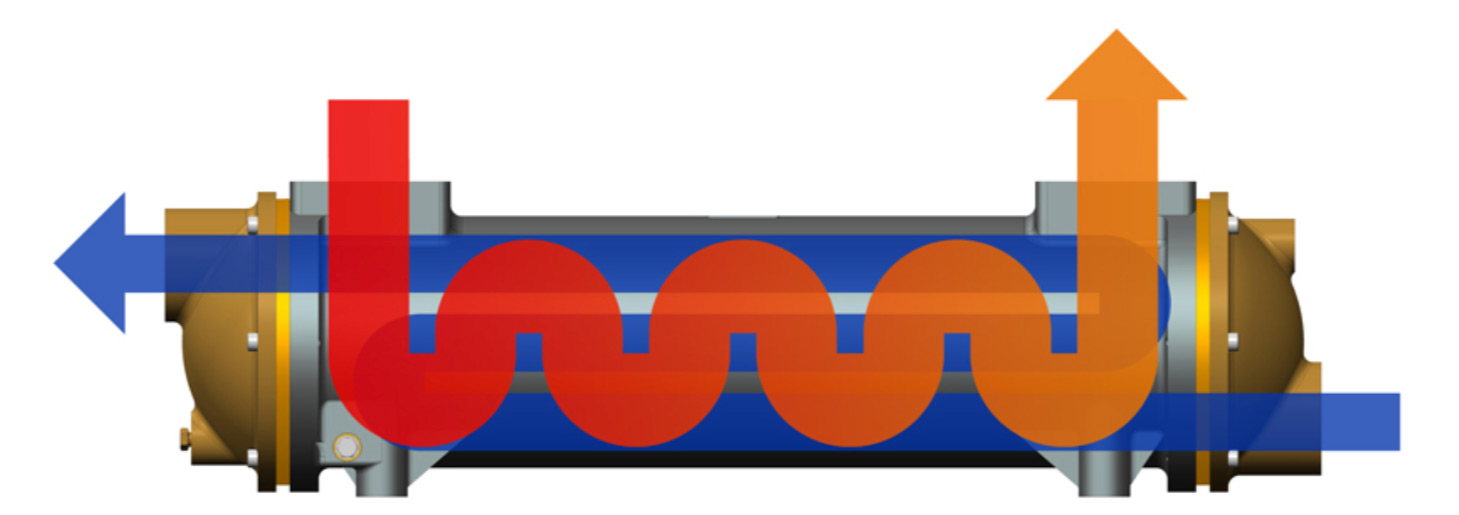
MSFD is common today; 60% of all desalinated seawater in the world[8] is produced through this method, and more than 18,000 MSFD facilities exist globally.[9] However, MSFD is an energy-intensive process with high operating expenses, making it more difficult to justify at larger scales. Scarcity Zero seeks to lower the cost of multi-stage flash distillation, giving us the ability to desalinate unlimited amounts of fresh water as a function of the framework.
The use of the word “unlimited” here bears special mention. There is a vastness to the oceans that “71% of Earth’s surface” does not give justice to. Only 0.4% of water on Earth is both fresh and accessible, and that’s been enough for humanity until the present. With that in mind, we would have to increase our water consumption thousands of times for desalination to even measurably impact sea levels – especially since the water cycle would eventually return all desalinated water to the ocean. And even if we did somehow lower sea levels, it would be to our benefit anyway, as sea levels are rising due to climate change.[10]
Valid questions exist as to the extent of the environmental impact of MSFD, both on local ecosystems and on the ocean as a whole. Conceptually, MSFD doesn’t do anything to the environment except place a pipe in the ocean and suck in seawater; rather than one large pipe that might risk capturing marine life, a smaller series of filtered pipes designed to reduce ecological impact can be used.
As these intake systems can operate twenty-four hours a day, a large volume of water can be secured through a slow yet steady flow – meaning it does not need to be strong enough to measurably interfere with the local ecosystem. The greatest environmental impact of MSFD plants today usually involves the dumping of waste brine back into the ocean with chemicals[11] – steps that need not be taken with modern facilities for two primary reasons:
- Chemical pretreatment of seawater is not as necessary in modern MSFD plants. Older models have sometimes introduced chemicals to “soften up” water, making it less corrosive and easier to heat, but modern polymers can resist corrosion[12] and Scarcity Zero provides ample inexpensive heat energy as a byproduct of power generation.
- Currently, some MSFD facilities pump waste brine back into the ocean, which raises local salinity levels and can cause environmental damage. With Scarcity Zero, we have plenty of excess energy to boil off waste brine and leave only salt as a byproduct.
That latter point presents an important question, though: if we were to desalinate seawater on a large scale, how do we deal with all the leftover salt? The answer? Simply sell it.
Let's say our implementation of Scarcity Zero desalinated a total of 500 billion gallons of water annually. Each gallon of seawater contains roughly 4.5 ounces of salt.[13] Therefore, 500 billion gallons of seawater would contain 2.25 trillion ounces of salt, or 140.63 billion pounds. That's a lot of salt – but our national salt consumption is equally high.
According to the U.S. Geological Survey, the United States consumed 69,500 thousand metric tons of salt in 2015 for all purposes.[14] At 69.5 million metric tons, that translates to 153.2 billion pounds of salt. This means a 500-billion-gallon annual desalination effort would yield around 91% of our annual salt consumption. At an estimated price of $40-$50 per long tonne (2,204lbs) 140.63 billion pounds would yield roughly $2.5 billion in profits from annual salt sales (assuming $40 per tonne).[15]
With these concerns addressed, MSFD technologies can be harnessed to produce unlimited amounts of fresh water for any use, with negligible fiscal and environmental costs. This would effectively end water scarcity as a concept. And we can then do the same for fuel.
Hydrogen Fuel
Hydrogen is the most abundant element in the universe.[16] It’s light, clean, and highly combustible, with an energy-per-mass ratio greater than any known fossil energy source.[17] This makes it a flexible and useful alternative to petroleum if we go about sourcing and storing it in the right way.
Hydrogen production is currently a $100+ billion industry,[18] yet most current methods of hydrogen production involve extracting the element from oil or coal through high-temperature steam reformation[19] – a process that is both environmentally destructive and will likely prove untenable once fossil fuels eventually become more scarce.[20] In a world with effectively unlimited cheap energy, electrolysis becomes a significantly more attractive method.
Electrolysis is a process that introduces an electrolyte and an electric current strong enough to break molecular bonds of water, chemically separating it into oxygen and hydrogen gas. Like Multi-Stage Flash Distillation, it’s not a new concept. Electrolysis has been in use since the 1700s to extract various substances, hydrogen among them.[21] Nor is it particularly complex; you could set up a simple facility in your garage, if you wanted to (just don’t smoke).
But to produce enough hydrogen for use as a viable fuel on a nationwide or global scale, an industrial setting would be necessary. Commercial hydrogen extraction through electrolysis has traditionally proven expensive,[22] but Scarcity Zero mitigates this cost factor as a byproduct of generating heat energy from thorium, making the production of hydrogen through electrolysis perfectly viable. Once extracted from water, hydrogen can be harnessed to power an array of systems and processes, to be discussed throughout the rest of this writing.
But even so, challenges to using hydrogen remain as production is only one half of the equation. The other is how to contain, transport and stabilize it – considerations of no small significance. Because hydrogen is highly reactive, it is easily contaminated as it naturally bonds to other substances.[23] And due to its volatility, it has usually required storage in containment tanks at high pressures. While metal tanks work in a laboratory or industrial setting, the weight of these tanks and the safety risks presented by the explosive nature of compressed hydrogen have made this approach questionable for civilian use. Thankfully, recent advancements have given us new alternatives, such as:
Graphene storage: Storing and transporting hydrogen in compressed form requires immense pressure, on the order of 482–690 bar (7,000-10,000 PSI).[24] Currently, this is only possible through metal tanks that have limited utility due to increased bulk and weight. Through Scarcity Zero, we’ll have better materials.
Although we’ll be reviewing materials further in Chapter Ten, one of the most noteworthy in the context of hydrogen is graphene,[25] which serves several important roles in the Scarcity Zero framework. Conceptually, graphene is a one-atom-thick sheet of carbon that is structured in a way that is both ultra-strong and ultra-conductive.[26] This allows graphene to both function as an efficient battery[27] and also a structural material – one that is 200 times stronger and six times lighter than steel.[28] As it can be made paper-thin while remaining flexible, graphene is well-suited to make storage tanks for hydrogen in vehicles and other machinery. Just as importantly, these storage mediums can be amorphously shaped, providing greater flexibility in how they integrate with a fuel supply.[29]
Synthetic oil: Scarcity Zero’s approach to solving resource scarcity is based in large part on replacing oil as a fuel source, due both to its finite supply as a fossilized product and its contributions to climate change. But oil has other important uses: it’s essential for making plastics and synthetic materials, and it's a critical ingredient for chemical engineering.[30] Oil is type of chemical known as a hydrocarbon, and hydrocarbons are useful for both organic chemistry and fuel for combustion. Oil is the abundant hydrocarbon of our time, so it’s what we use. But that doesn’t have to be the case, especially as oil eventually becomes increasingly scarce and thus expensive in the future.[31]
With an abundant supply of hydrogen, we can use it to manufacture synthetic hydrocarbons for lubricants and chemical stabilizers[32] as well as specialized fuels for sophisticated applications like aircraft and rockets.
We can also use synthetic hydrocarbons for long-term hydrogen storage. Once processed into a solution that stays liquid at normal pressure, we can store an effectively unlimited amount of hydrogen in tanks that don’t require compression and would work similarly to how we transport liquid fuels today. And unlike the environmental damage caused by crude oil drilling, refining and transport, synthetic oil can be processed at facilities that remove ecologically hazardous steps from the manufacturing chain. This becomes all the more important when using hydrogen as a medium to create fuel from atmospheric carbon capture. (We’ll go over these details next chapter).
Fuel Cells: A hydrogen fuel cell is a means of generating electricity from a chemical fuel, in this case, hydrogen. In practice, this allows fuel cells to function as emission-free batteries. Fuel cell technology has been around since the 1950s and has steadily grown since then into a billion-dollar market,[33] with several proven designs powering myriad industrial applications.
In a dynamic with reduced energy costs and improved manufacturing processes, fuel cells become less expensive, easier to build and easier to expand into varied sectors of our economy.
Although hydrogen fuel cells are often looked to as a replacement for oil, they also have potential to power remote areas that are environmentally hostile to power generation. There are several circumstances where it’s not feasible to rely on local power systems and where solar isn't possible (war/disaster zones, remote research facilities, long-voyage ships, space travel, etc.), yet fuel cells can provide energy as long as a supply of hydrogen exists. Future advances in graphene battery technology can also complement this possibility, allowing for robust energy storage even when far away from civilization’s amenities.
Adding Things Together
With municipally integrated renewables doing their part to power cities and reduce the energy demand they place on regional electric grids, employing the baseload electricity generation and excess heat energy of LFTRs to both desalinate seawater and extract hydrogen becomes a routine deliverable of the framework. Yet that merely reflects only the products of this approach – its underlying strategic value is itself a step further. As we’ve discussed previously, a key component of Scarcity Zero is the intention to deploy energy technologies strategically so that they can work together as a team to become greater than the sum of their parts in operation.
The next stage of this goal comes through conceptual “CHP Plants” – (Combined Heat and Power) – which are modular arrays of the technologies thus-far discussed that can be installed rapidly in varied configurations for specific applications. As we’ll see later in this writing, these applications might include atmospheric scrubbing of greenhouse gasses, waste processing and recycling, large-scale ocean cleanup or supplemental energy and resource production. Yet they each would deliver their intended goals as a system that’s standardized and cogenerative in design, enabling turnkey deployment of energy generation and resource production to solve most any problem caused by the absence of either.